Project 3 PMC/NLC altitude, frequency and brightness changes related to changes in dynamics and chemical composition
From CAWSES
- Project leaders: G.Thomas (USA), U.Berger (Germany)
- Project members: S. Bailey (USA),G. Baumgarten(Germany),M. DeLand (USA), J. Fiedler (Germany),B. Karlsson (Sweden), S. Kirkwood (Sweden), A. Klekociuk (Australia), F.-J. Lübken (Gemany), A. Merkel (USA), N. Pertsev (Russia), J. Russell III (USA), E. Shettle (USA)
Introduction
The 20-yr old speculation that high-altitude summertime ice clouds (polar mesospheric clouds or noctilucent clouds, here denoted MC) are affected by anthropogenic activities has recently received support from a 30-year time series of SBUV (Solar Backscatter Ultraviolet) satellite measurements (see Figure 1). SBUV data reveal a significant trend in bright MC properties. However, the robustness of the trend, extracted from interannual, local-time and solar-cycle variability, and its underlying causes remains debatable. It is important to understand the relative roles of these three factors (solar, inter-annual and long-term forcing) before a definitive long-term trend can be evaluated. Furthermore the problem of attribution, that is, the nature of the various forcings on ice formation is not yet understood. For example with respect to the long-term changes, is a lowered temperature due to higher carbon dioxide responsible for the observed increase in brightness and occurrence frequency of MC? Or are water vapor changes due to oxidation of methane responsible, since we know that lower atmospheric methane has more than doubled over the past 120 years, and methane oxidation leads to upper atmospheric water vapor. The failure to detect any changes in the altitude of MC since the first measurement made by Otto Jesse in the late nineteenth century has provided an important constraint, since water vapor changes and temperature changes affect cloud altitude in different ways. Fortunately, the state of the art in modeling has now reached a point where ice formation is coupled with general circulation models. In a recent study Berger and Lübken (2011) showed that in the summer period 1979 -1997 at mid-latitudes strong cooling of up to 3-4 K/decade occurs in the middle mesosphere, in the period 1961-1979 the middle atmosphere cooled significantly less, and for the period 1997-2009 they find a warming of ~1 K/decade. For the first time, modeled temperature trends confirm the extraordinarily large temperature trends observed at mid-latitudes during the period 1979-1997 derived from lidar measurements, satellite data, and phase height measurements. The differences in temperature trends in the mesosphere originate from the evolution of stratospheric ozone in the past 50 years, e.g. the observed reversal of both stratospheric and mesospheric temperature trends in the mid 1990s is caused by the recovery of stratospheric ozone (WMO report 2011). Therefore a new research question arises: does any trend in MC show a similar behavior? Relevant publications on this subject are reported in the references below:
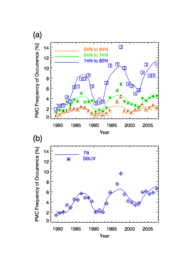
References
- Luebken, F.-J., U. Berger, and G. Baumgarten (2009), Stratospheric and solar cycle effects on long-term variability of mesospheric ice clouds, J. Geophys. Res., 114, D00I06, doi:10.1029/2009JD012377
- Merkel, A. W., Marsh, D. R., Gettelman, A., and Jensen, E. J.: On the relationship of polar mesospheric cloud ice water content, particle radius and mesospheric temperature and its use in multi-dimensional models, Atmos. Chem. Phys., 9, 8889-8901, 2009
- Merkel, A. W., D. Marsh, G. E. Thomas, C. Bardeen, M. Deland, WACCM simulations of long-term changes in polar mesospheric clouds, Layered Phenomenon in Mesospheric Regions (LPMR) Conference, Stockholm, 2009
- Marsh, D and A. W. Merkel, 30-year PMC variability modeled by WACCM, SA33B-08, Fall AGU Meeting, San Francisco, 2009
- Shettle,E. P.,M. T. DeLand, G. E. Thomas, and J. J. Olivero (2009), Long term variations in the frequency of polar mesospheric clouds in the Northern Hemisphere from SBUV, Geophys. Res. Lett., 36, L02803, doi:10.1029/2008GL036048.
- Thomas, G. E., D. Marsh and F.-J. Lübken, Mesospheric ice clouds as indicators of upper atmosphere climate change, EOS, Transactions, American Geophysical Union, 91, No. 20, 18 May 2010, p. 183.
- Berger, U., and F.-J. Lübken (2011), Mesospheric temperature trends at mid-latitudes in summer, Geophys. Res. Lett., 38, L22804, doi: 10.1029/2011GL049528.
- WMO (2011), Global ozone research and monitoring project-Report no. 52, Scientific assessment of ozone depletion: 2010, World Meteorological Organization
Workshops and Meetings
The first workshop under the auspices of this working group was held in Boulder, Colorado on December 10 & 11, 2010, entitled Modeling Trends in mesospheric clouds (Thomas et al, 2010).
Second CAWSES-2 Task 2 Workshop: Modeling Polar Mesospheric Cloud Trends, May 3-4, 2012
Laboratory for Atmospheric and Space Physics, University of Colorado, USA.
This is the 7th workshop sponsored by IAGA/ICMA/CAWSES and focusses on global change in the upper mesosphere, specifically decadal-scale trends in Polar Mesospheric Clouds, PMC (or Noctilucent Clouds, NLC, as they are traditionally called when observed from the ground at twilight).
The issue of long-term changes in PMC was addressed in the first workshop in Boulder, Colorado, 10-11 December, 2009 (Thomas et al, 2010). A long suite of satellite measurements by the Solar Backscatter Ultraviolet Spectrometer (SBUV) dating back to 1979 shows a significant long-term trend in mesospheric cloud activity and brightness. Attribution of trends in these high-altitude ice clouds (~83 km) includes: (1) increasing carbon dioxide concentrations which tend to cool the upper mesosphere (the 80-90 km 'mesopause region'); (2) increasing water vapor due to growing concentrations of methane, in addition to changing efficiency of tropospheric water vapor entry through the tropopause; (3) shrinking of the upper atmosphere arising largely from decreased ozone levels and subsequent stratospheric cooling; (4) increased concentrations of water vapor due to the rise in space traffic over the last 30 years. Other possible causes are long-term changes in winds and wind filtering of gravity waves which dominate the dynamics of the high-latitude summertime mesopause region. An even larger (cyclic) trend is superimposed on the long-term record, believed to be a result of the solar cycle, but not well understood.
General circulation models coupled with ice formation are now capable of simulating the SBUV trends to a remarkable degree, as discussed in the first workshop. However, new modeling results have brought out the importance of ozone trends, which affect the heat balance of the entire upper atmosphere. The LIMA/ICE model predicts a 'break-point' in the time series of PMC trends, due to the turn-around of the ozone trend in the mid-1990's. A wealth of new data from several satellite missions (AIM, ODIN, TIMED) are available. The Aeronomy of Ice in the Mesosphere (AIM) directly addresses the processes which control ice cloud evolution. In addition, more information is now available on diurnal variations in ice water content, which in principle affects the analysis of trends made from satellites in sun-synchronous orbits with slowly-varying local time coverage. These and other advances in modeling, data availability and trend analysis are motivations for a coming together of both modelers and data analysts to assess the current state of knowledge. A goal of the workshop would be to make recommendations for future work to resolve many of the outstanding issues.
The second PMC Trend Workshop was held at the Laboratory for Atmospheric Physics at the Space Science Building, the newest LASP location at the University Research Campus Park, University of Colorado, Boulder, Co, USA on May 3 and 4, 2012. Short abstracts of the talks (where available) are given below on this WIKI page.
Gary Thomas (thomas@lasp.colorado.edu) and Uwe Berger (berger@iap-kborn.de), co-chairs of CAWSES-II, Task 2, Project 3
TITLES, ABSTRACTS OF PAPERS GIVEN AT THE WORKSHOP. THE PDF AND AUDIO (MP3) FILES ARE AVAILABLE FOR DOWNLOADING.
YOU CAN NOW SEE AND HEAR THE PRESENTATIONS, BY CLICKING ON THE INDIVIDUAL LINKS. IT IS ADVISED TO FIRST DOWNLOAD THE PDF FILE, AND SAVE IT. THEN DOWNLOAD THE MP3 FILE, WHICH WILL START IMMEDIATELY. YOU CAN SAVE ALL THE FILES YOU WANT FOR LATER VIEWING, BUT THEY NEED TO BE DOWNLOADED INDIVIDUALLY.
Gary Thomas, LASP,USA, Welcome, Background and CAWSES Objectives[2]
A brief description is given of the Task Group 2, the members of the steering committee, and the outcome of the first workshop
Long-term Trends: PMC Observations
John Olivero, Embry-Riddle University, USA, Some Historical Notes on Noctilucent Cloud Studies[3] Audio file [4]
We discuss some of the highlights of the history of noctilucent cloud studies beginning in the 1880's. A second way to look at this history is by casting it in terms of a personal scientific journey. Many unsolved problems exist.
Matthew DeLand, SSAI, USA, Current PMC Trends Derived from SBUV Measurements[5] Audio file [6]
SBUV instruments provide measurements of PMC's in both hemispheres extending from 1978 to the present. Combining data from eight separate instruments for trend analysis requires careful treatmnet of effects that depend upon scattering angle and local time. The magnitude and latitude dependence of long-term PMC trends derived from SBUV data are sensitive to the analysis methodology.
Mark Zalcik, Coordinator, NLC Can Am Network, Canada, Two Decades of Noctilucent Cloud Monitoring in North America[7] Audio file [8]
There is a data set of ground-based visual NLC observations in North America to complement those of Russia and Western Europe. The NLC CAN AM network started in 1988 and we have been collecting observational data from amateur weather watchers and astronomers as well as from weather and flight service stations across Canada and the U.S. Our twenty-four consecutive years of monitoring has rendered a compelling activity curve with regard to active NLC nights. We have devised a statistical treatment of tropospheric cloud conditions recorded during NLC sky checks and have applied the derived equation to selected observations at the La Ronge, Saskatchewan Flight Service Station. The results appear to show that at least half of NLC displays are being missed due to tropospheric weather. We corroborated the global low-point of activity in 1992; however, at the following solar maximum in the mid-2000's, NLC activity remained high. Of special interest has been the regular sightings of encroaching low-latitude bright NLC at mid-season, with sightings from places such as the states of Oregon, Utah, Colorado and New York. At present, the frequency of such low-latitude displays is 1-2 per year. It will be interesting to see if this frequency changes in the future. In 2010, our observations indicated NLC in general did not drift as far south as they normally would. The situation requires more study. We have found that the more observers, the more active nights we tabulate, suggesting that NLC are of a patchy nature with many nights experiencing NLC over small areas. Hence it would be desirable to have even more eyes looking up from the ground, and on that note, we look forward to more participation.
P. Dalin, Swedish Institute of Space Physics, Sweden,On the long-term trends in noctilucent clouds as observed from the ground and on the trends in the OH summer temperature as measured in Moscow and Lithuania[9] Audio file [10]
We report on the long-term trends in noctilucent clouds (NLCs) as observed from Moscow and Lithuania and on the trends in the OH summer minimum temperature as measured in Moscow. The Moscow NLC characteristics are analyzed for the period from 1962 to 2011 (for 50 years). The Lithuanian NLC data sets are considered for the periods from 1973 to 2011 and from 1991 to 2011. The OH minimum summer temperatures are measured with a digital spectrograph located near Moscow, which allows us to measure the OH rotational temperature at around 87 km above latitudes of 56-57°N. We demonstrate that there exists a slight negative trend (-0.35 K/yr) in minimum temperatures around the summer mesopause from 2000 to 2011. However, the trend lacks statistical significance. There is no statistically significant long-term trend in the Moscow NLC observations for 50 years (the period is longer than any satellite time series of PMCs). This is valid both for time series of the NLC number and NLC brightness estimations. The statistical analysis of the Lithuanian data sets show that there is a slight long-term positive trend (0.11 N/yr) in NLC observations of all brightness from 1991 to 2011 as well as in very bright NLCs (0.04 N/yr) from 1973 to 2011. However, both trends lack statistical significance. Our OH summer temperature measurements can be used as the input for PMC/NLC modeling at latitudes of 56-57°N. It would be worth running a PMC model for latitudes of 57-60°N for which we have reliable long-term NLC data. Our NLC data sets could be simulated in PMC models.
Gerd Baumgarten, IUP, Germany,Decadal observations of particle sizes and water vapor content of NLC[11]
Audio file:[12]
Observations of particle properties in noctilucent clouds have been performed with the ALOMAR RMR-Lidar in northern Norway throughout the last decade. The observations reveal a complicated interplay of number density, particle size and distribution width throughout the vertical structure of the clouds. Year to year variations of number density are huge (factor of 5) but are compensated by size and width variations so that the resulting changes of the water column in NLC is less than 20%. Taking the occurrence frequency of the clouds into account gives a good agreement to zonal mean ice mass deduced from the LIMA model
Long-term Trends: Temperature & Water Vapor Observations
Alain Hauchecorne, Laboratoire ATmosphères, France, Temperature trends in the stratosphere and in the mesosphere as seen from Rayleigh lidar observations[13] Audio file [14]
This presentation concerned the evaluation of trends and variability of temperature profiles measured at the Haute-Provence Observatory in France. The observations apply to both the stratosphere and mesosphere. Comparison is made with the satellite measurements of stratospheric temperature trends from SSU and AMSU. The summertime data show slight negative trends at all altitudes, consistent with radiative cooling from CO2 increase. Winter data show the importance of taking into account some possible changes in the occurence of sudden stratospheric warming events. This presentation showed also the need to take into account the effect of tides for the trend analysis of satellite temperature data, which apply only to specific local times.
Karen Rosenlof, NOAA, USA, A new satellite based zonally averaged time series of stratospheric water vapor[15] Audio file [16]
Gerald Nedoluha, NRL, USA, Long-Term Ground-based Microwave Measurements of Middle Atmospheric Water Vapor from NDACC sites[17] Audio file [18]
Michael Stevens, NRL, USA, The impact of space shuttle main engine exhaust on PMCs and implications to trend studies[19] Audio file [20]
From the final launch of the Space Shuttle in July, 2011, we find that the main engine plume formed PMC brighter than 99% of all other PMC's. Since SBUV measures only the brightest PMC, we compare the the total water vapor exhaust input to the upper atmosphere to the ice mass observed by SBUV. We find that between 1979 and 2011, the water exhaust available from space traffic is, on average, four times larger than the ice mass observed by SBUV at latitude 70N (plus/minus 2.5 deg).
Long-term Trends: Modeling
Uwe Berger, IAP, Germany,Solar variability and trend effects in mesospheric ice layers [21] Audio file [22]
The atmospheric shrinking due to atmospheric cooling enhances mesospheric cloud trends, through temperature effects. PMC trends are non-monotonic. The appearance of break points in PMC trends in 1978 and 1997 appear to be due to similar behavior of stratospheric ozone evolution.
Aimee Merkel, LASP, USA, WACCM-PMC simulations of long-term trends of PMC[23] Audio file [24]
A summary overview of WACCM-PMC modeling of PMC trends is presented. Highlighted was the sensitivity of the PMC trend to the brightness (albedo) threshold. No trend in dim clouds is found. Also we emphasize the importance of attribution studies to determine the sensitivity of PMC trends to greenhouse gas trends.
David Siskind, NRL, USA,'The PMC region as an integrator of coupling processes: Implications for trend studies from AIM and other missions '[25] Audio file [26]
Stan Solomon, NCAR, USA,Thermospheric Temperature Trends: Modeling and Observations [27] Audio file [28]
Dan Marsh, NCAR, USA, Climate change in the mesosphere from 1850 to 2100 in CESM-WACCM [29] Audio file [30]
Kota Okamoto, The University of Tokyo, Japan, On the dynamical responses in the middle atmosphere to ozone recovery and CO2 increase [31] Audio file [32]
Inter-annual, hemispheric and seasonal variability: Observations
James Russell III, Hampton Univ, USA, AIM science results and their significance for PMC long-term change studies [33] Audio file [34]
Cora Randall, LASP, USA, AIM/CIPS Observations of PMC Variability [35] Audio file [36]
This talk summarized the AIM/CIPS data that are publicly available, and described some of the PMC variations that have been observed by CIPS. The current data version is v4.20r05; anyone interested in the data should visit http://lasp.colorado.edu/aim/. Available data include orbit-by-orbit retrievals of cloud albedo, particle radius, and ice water content from May 2007 to the present, at 25 km^2 spatial resolution. The season-average variation in frequency compared to average temperatures and water vapor derived from the MLS instrument were highlighted. It was shown that there was a strong anti-correlation (correlation) with temperature (water vapor) except for the SH 2009-2010 season. The first two months of that season were anomalous, at least in part because of dynamical coupling with the SH stratosphere below, and higher mesospheric water vapor than in the other years. Intra-seasonal variations were also described; inter-hemispheric teleconnections are evident in PMC data from both hemispheres. We showed that there are interesting asymmetries in the spatial distribution of the clouds that persist even when averaged over an entire month; these are presumably driven by asymmetries in the gravity wave distribution. A simple equilibrium model can explain the overall variations from season to season, but more detailed quantitative analyses are needed. We also showed that the bright-cloud frequency varies inversely with the dim-cloud frequency in the middle of the season, a relationship which also holds for the SNOE and OSIRIS data, suggesting a systematic conversion between large and small particles
Rachel Ward, Utah State University, USA, Comparison of Northern and Southern Hemisphere Mesospheric Gravity Waves using CIPS PMC Data [37] Audio file [38]
Susanne Benze, LASP, USA, On the onset of polar mesospheric cloud seasons [39] Audio file [40]
Dynamical effects, for example from the Antarctic ozone hole or from changes in the wind or temperature distribution from a warming stratosphere, should be taken into account when analysing trends in PMC's. This is in addition to chemical effects from increasing CO2 & CH4. It is possible that these two influences interact. The southern hemisphere PMC date of onset is strongly influenced by intra-hemisphere coupling with lower atmospheric stratospheric winds. This coupling exists due to seasonally-changing zonal wind flow which filter gravity waves differently as the wind changes from winter to summer. The breaking of the the gravity waves, and its variation with season, forces changes in the residual circulation, which affects temperature and hence PMC onset. A deepening ozone hole results in a trend towards later onset of the PMC season in the southern hemisphere. With the recovery of the ozone hole expected in the next 4-5 decades (due to the Montreal protocol banning of CFC's), it is expected that the dynamical component will cause earlier PMC onset dates in the future. This would proceed in the same direction as the chemical trend from CO2 cooling and enhanced H2O from increasing CH4.
Hanli Liu, NCAR, USA,Fast meridional transport in the lower thermosphere by planetary-scale waves [41] Audio file [42]
We study the fast meridional transport of STS-107 Columbia Shuttle plume in January 2003 at 100-115 km. Diagnostic calculations are performed to trace the particle trajectories using winds from the Thermosphere-Ionosphere-Mesosphere-Electrodynamics General Circulation Model (TIME-GCM) simulations for January, when the amplitude of the quasi-two-day wave (QTDW) usually peaks. These simulations thus suggest that the observed rapid planetary-scale meridional transport of the shuttle main engine plume can be driven by planetary waves and tides. We also demonstrate that the rapid eastward propagating STS-121 Shuttle plume in July 2006 is mainly driven by the zonal wind jet above the mesopause when the planetary waves are weak. The contrast of the two demonstrates the seasonal dependence of shuttle plume transport, which further implicated roles of mean circulation and waves in the mesosphere and lower thermospheric region transport.
Properties of PMC and their Environment: Observations
E.J. Llewellyn, University of Saskatchewan, Canada], Special Observational Opportunities Offered by PMCs - Nadir Observations in the Limb [43] Audio file [44]
Scott Robertson, U of Colorado, USA, Detection of Meteoric Dust in Mesosphere by the CHAMPS Rockets [45] Audio file [46]
Kristell Pérot, LATMOS Laboratoire ATmosphères, France, PMC Particle Size Retrieval from GOMOS / ENVISAT Observations [47] Audio file [48]
I have presented the main results obtained on PMC from GOMOS/ENVISAT instruments. This work leads to a very large data set. We observed 21,000 PMC in both hemispheres over a 10-year period. This very rich data set calls for detailed study, as GOMOS offers some unique advantages over other satellite observations. In particular, it is the only space-borne instrument which provides measurements at different local times, due to the technique of stellar occulations. The accompanying photometric observations, pointing in a variety of directions over the full diurnal cycle address a very important issue for understanding PMC trends
Properties of PMC and their Environment: Modeling
Charles Bardeen, NCAR, USA, Simulations of PMC during the AIM time period using WACCM/CARMA [49] Audio file [50]
Simulations of PMCs from a free running version of the Whole-Atmosphere Community Climate Model (WACCM) coupled with sectional microphysics from the Community and Radiation Model for Atmospheres (CARMA) for meteor smoke and cloud ice yields results that are in very good agreement with observations from the Solar Occultation for Ice Experiment (SOFIE) and the Cloud Imaging and Particle Size experiment (CIPS). Improvements in the simulation of ice number density can be shown by increasing the temperature variability caused by subgrid scale gravity waves that is not resolved by the model. Initial and preliminary results are shown using the latest version of WACCM/CARMA nudged to a specified meteorology for the AIM time period. Seasonal averages are similar to observation and previous simulations, but with a different seasonality. These differences appear related to the introduction of a more physically based gravity wave scheme in WACCM4 that is just now being tuned for use with PMC models.
Mark Hervig, GATS, Inc., Variability in PMCs and their environment from SOFIE observations, and potential implications for PMC trends [51] Audio file [52]
SOFIE data in conjunction with a "zero-D" model indicate that the bright PMC's observed by SBUV are ~ 3 times less sensitive to water vapor changes and ~5 times less sensitive to temperature changes, compared with the total PMC population. Using SOFIE and the model to relate PMC changes to temperature, the SBUV PMC albedo trend (currently ~2%/decade) corresponds to a temperature trend of -0.5 to -1.0K/decade
I. Azeem, Astraspace, USA, Simulations of Shuttle Main Engine Plume Effects on Lower Thermosphere Energetics and Chemistry [53] Audio file [54]
Panel discussion, J. Russell III, J. Olivero, E. Shettle, U. Berger, M. Stevens Audio file [55]
SPECIAL JOURNAL ISSUE ON UPPER ATMOSPHERIC TRENDS NOW AVAILABLE. The joint JGR/Space, JGR/Atmospheres special section on upper atmospheric trends is now complete, and can be accessed at http://www.agu.org/journals/ja/special_sections.shtml?collectionCode=UATREND1&journalCode=JA
Observing Facilities
Aeronomy of Ice in the Mesosphere (AIM), a NASA satellite mission (2007-)
AIM was launched from Vandenberg Air Force Base on April 25, 2007 becoming the first satellite mission dedicated to the study of Polar Mesospheric Clouds (PMCs). A Pegasus XL rocket placed the AIM satellite into a near circular (601 km apogee, 595 km perigee), 12:00 AM/PM sun-synchronous orbit. By measuring PMCs and the thermal, chemical and dynamical environment in which they form, AIM will quantify the connection between these clouds and the meteorology of the polar mesosphere. In the end, this will provide the basis for study of long-term variability in mesospheric climate and its relationship to global change. The results of AIM will be a rigorous test and validation of predictive models that then can reliably use past PMC changes and current data to assess trends as indicators of global change. This goal is being achieved by measuring PMC densities, spatial distribution, particle size distributions, gravity wave activity, meteoric smoke influx to the atmosphere and vertical profiles of temperature, H2O, O3, CH4, NO, and CO2.The overall goal of AIM is to resolve why PMCs form and why they vary. It has been suggested that the observed changes in the clouds are related to increased concentrations of greenhouse gases. This suggestion is plausible because an increase in carbon dioxide, while warming the surface of the Earth, cools the upper atmosphere which can facilitate mesospheric cloud formation there. Additionally, increases in methane at the surface of the Earth lead to increases in water vapor at high altitudes through chemical oxidation processes, which further facilitates cloud formation and growth. While plausible, this greenhouse gas hypothesis has not yet been proven.The AIM webpage is at [56]
Arctic Lidar Observatory for Middle Atmosphere Research (ALOMAR)
Fig. 2:Year-to-year variability of seasonal mean NLC occurrence and altitude for two different cloud classes. The blue curves contain all measurements having a sensitivity above the long-term detection limit, whereas the green curves show results for strong clouds only. The vertical bars indicate 95% confidence limits for the occurrence and errors of the mean altitudes. For more information see text and references.The Rayleigh/Mie/Raman (RMR) lidar at the Arctic Lidar Observatory for Middle Atmosphere Research (ALOMAR) is located on top of a ≈400 m high mountain in Northern Norway (69.3°N, 16.0°E). It was installed in 1994 and designed for multi-parameter investigations of the Arctic middle atmosphere on a climatological basis. Because of its Arctic location, the lidar is optimized for measurements during full sunlight. Appropriate technical solutions, laser wavelength stabilization in combination with strong spectral as well as spatial filtering at the receiving system, have been implemented. The lidar is a complex twin-system consisting of two power lasers, two receiving telescopes, and one optical bench for spectral separation and filtering of the light received from the atmosphere. The lasers emit pulses at three wavelengths (355 nm, 532 nm, 1064 nm) simultaneously with an overall peak power of 150 MW per laser. The backscattered light from the atmosphere is collected by telescopes with a diameter of 1.8 m each. They can be tilted up to 30° off-zenith to allow different viewing directions (Fig. 1), which separates the sounding volume up to 90 km at an altitude of 80 km. After spectral and intensity separation the light is analyzed by 15 channels and recorded by single photon counting detectors. One main objective of the RMR-lidar is the observation of ice layers in the mesopause region, which are known as noctilucent clouds (NLC) and polar mesospheric clouds (PMC). Throughout the NLC season (1 June to 15 August) the lidar is operational for 24 hours per day to measure whenever permitted by the local weather conditions. This yielded a total of more than 4000 measurement hours from 1997 to 2009. NLC were observed during ≈1700 hours which is the largest NLC data base acquired by lidar. The data are used to investigate decadal scale changes of NLC parameters, size and number density of the ice particles, as well as small scale structures which are often observed in the cloud layers. Fig. 2 shows the time series of NLC occurrence and altitude above ALOMAR covering one solar cycle (taken from reference 2). During these 11 years there is no statistical significant anti-correlation between cloud occurrence and solar activity, which is partly in contrast to other data sets. The mean cloud altitude is 83.2 km and appears to remain nearly unchanged since the first NLC observations more than 100 years ago.
Visual Observations of Noctilucent Clouds
Scientific interest in noctilucent clouds (NLC) can be traced back to 1884 when many observers watched the twilight sky to see the dramatic sunsets caused by dust from Krakatoa, which had erupted during the previous year. Captivating displays of 'night shining' clouds, were seen and quickly recognized as lying at much higher altitude than normal clouds. For much of the 20th century, visual and photographic observations were the only methods available for systematic monitoring of NLC characteristics. By the early 1960's it was clear that their occurrence rates varied enormously from year to year. Widespread, intense displays of NLC were seen for a few consecutive summers, with NLC reported from somewhere around the 50 - 60 N latitude band almost every night over the summer season. These periods were followed by years when almost no NLC at all were seen. The most notable 'high spots' for NLC were the years 1885-1890 and 1963-1968, which were each followed by strong declines in NLC reports even though the same observers as during the 'hot spots' continued to look for them (reference 1).Starting with the International Geophysical Year in 1958, professionally organized observing networks started to gather systematic records of visual sightings of noctilucent clouds (see references). Even though professional involvement has generally ended, or become sporadic, systematic NLC observations are still collected by networks of enthusiasts, in Europe, Russia, Canada and North America. Since 1996, visual observations by members of the public are collected at a number of regional or national centers [57], and since 1996 at the 'Noctilucent Cloud Observers Homepage' [ http://www.kersland.plus.com/]. NLC observations from the UK and Denmark since 1964 form the longest continuous record (observing latitudes from 51 - 61 N). These show how NLC are most common in years of low solar activity, and rare in years of high solar activity. They do not show any significant increase over the last 45 years although a few percent increase (or decrease) cannot be ruled out (Fig. 2, reference 3).
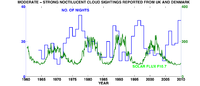
Although monitoring of large-scale structures and possible trends in NLC is being taken over by satellite measurements, visual and photographic observations still have an important role to play. For example, they are the best method available for studying fine-scale structure, on scales of 10s of km or less, and they can be instrumental in identifying NLC at unusually low latitudes where satellites observations and ground-based remote sensing instruments are not available.
- Fogle,B.and B.Haurwitz,Long term variations in noctilucent cloud activity and their possible cause, in Climatological Research,edited by K.Fraedrich, M.Hantel,H.Claussen Korff,and E.Ruprecht,pp. 263–276,Heft 7,Bonner Meteorologische Abhandlungen.,Bonn,Germany,1974.
- Romejko, V.A., P.A. Dalin, N.N. Pertsev, Forty years of Noctilucent Clouds observations near Moscow: database and simple statistics, J. Geophys. Res., 108, D8, 8443, doi: 10.1029/2002JD002364, 2003.
- Kirkwood, S., P. Dalin, A. Rechou, Noctilucent clouds observed from the UK and Denmark – trends and variations over 43 years, Annales Geophysicae, 26, 1243-1254, 2008.
Additional notes about NLC long-term behavior according to visual observations
1. There is no distinct contradiction when comparing a trend in the NLC occurrence frequency from ground-based observations and a trend in the PMC satellite observations for lower latitudes (50-64°N), if we take the same years for an analysis. The difference between the NLC and PMC trend is rather in their significance probability. However, if we consider ground-based NLC observations for more than 40 years (which are longer than space-borne measurements) we arrive at almost zero trend in the NLC occurrence frequency and at very small positive trend in the NLC brightness which have no statistical significance.
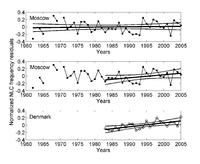
2. NLC most frequently occur in 1-2 years after the sunspot minimum and this delay is statistically significant. The PMC observations show a smaller delay of 0.5±0.5 year.
3. Concerning the year of the NLC discovery, we should note the following. The majority of papers, devoted to first observations of noctilucent clouds, refer not to 1884 but to 1885 as a year of first reliable descriptions undoubtedly concerning noctilucent clouds. Leslie (1884) did describe some sky phenomenon, resembling noctilucent clouds, but in his successive papers, Leslie (1885) and (1886), devoted to luminous clouds as a new phenomenon, he wrote nothing about priority of that observation of 1884; moreover, he did not refer to it at all. So, the description of Leslie's observation of 1884 cannot be regarded as reliable. Summarizing, Gadsden and Schröder (1989) wrote in their canonical book: “…It is certain that at the times of coloured twilight appearances of 1883/1884, no noctilucent clouds were discovered. Various reports also exist which could be interpreted as noctilucent clouds, but this will always remain uncertain (Pernter 1889; Schröder 1975; Gadsden 1985).”.
References:
- Dalin, P., S. Kirkwood, H. Andersen, O. Hansen, N. Pertsev, V. Romejko, Comparison of long-term Moscow and Danish NLC observations: statistical results, Annales Geophysicae, 24, 2841-2849, 2006.
- Leslie, R., 1884. The sky-glows. Nature 30, 583.
- Leslie, R., 1885. Sky glows. Nature 32, 245.
- Leslie, R., 1886. Luminous clouds. Nature 34, 264.
P. Dalin, N. Pertsev